Warning message
- Last import of users from Drupal Production environment ran more than 7 days ago. Import users by accessing /admin/config/live-importer/drupal-run
- Last import of nodes from Drupal Production environment ran more than 7 days ago. Import nodes by accessing /admin/config/live-importer/drupal-run
Unpublished Opinions
Unpublished.ca is a web portal on politics and current affairs in Canada. It provides the opportunity for Canadians to dig deeper into the issues affecting them, and to weigh-in on these issues in a persuasive and respectful way. Join the movement and have your say today!
The Intensification of the Water Footprint of Fracking
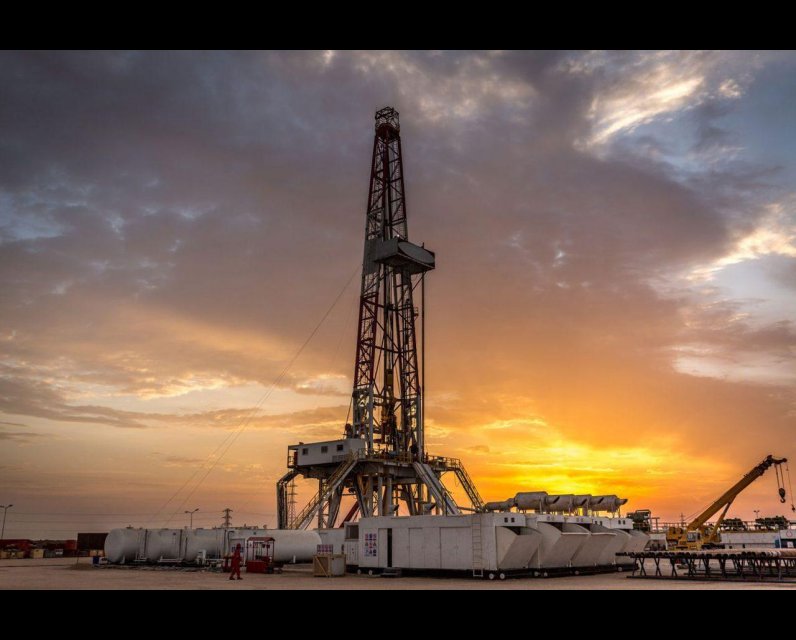
Authored By: Andrew J. Kondash, Nancy E. Lauer, Avner Vengosh*
*Corresponding author. Email: vengosh@duke.edu
From ScienceAdvances;
http://advances.sciencemag.org/content/4/8/eaar5982.full
INTRODUCTION
The environmental impacts of a fossil fuel–powered economy have led many nations across the world to begin developing greener energy and transportation solutions. In particular, the water footprint of fossil fuel exploration and electricity production has been projected to have major environmental impacts. It has been estimated that global water withdrawal for energy production constitutes 15% of the world’s total water consumption (1). Rapidly diminishing global water resources due to population growth and climate change have further exacerbated energy dependence on water availability, particularly in water-scarce regions (2–5). The beginning of the 21st century marks a special era with respect to global energy and water resources. The development of new drilling technologies and production strategies such as horizontal drilling and hydraulic fracturing has significantly improved the production of natural gas and oil by stimulating fluid flow from impermeable shale rocks previously not considered viable energy sources. Since the mid-2000s, these developments have spurred exponential growth of unconventional gas and oil well drilling across the United States and are spreading now to other parts of the world (Figs. 1 and 2) (4, 6–10). The rise of unconventional energy development has generated public debate on its environmental implications (11–16), especially with respect to both water availability and quality (2, 4, 8, 17–21).
Fig. 1 - Map of water stress and shale plays.
(A) Map showing the global water stress overlaid with shale formations across the world. (B) Water stress and shale regions in the United States examined in this study (5, 10).
Fig. 2 - Box plots of water use with lateral lengths.
Water use per well data (cubic meter per well; left y axis) for shale gas (top row) and tight oil regions (bottom row) with median lateral lengths per well (meter per well; right y axis) for each region plotted as colored lines. The central line of each box is the median, while the top and bottom of each box represent the third and first quartile, respectively. Whiskers on the box plot represent maximum and minimum values, while circles above the box plots show outliers in the data. Whiskers on the colored lateral length lines show the 95% bootstrap confidence intervals.
The process of hydraulic fracturing uses large volumes of water mixed with chemicals and proppant (sand) to fracture and hold open fractures in low-permeability shale and tight oil rocks to allow extraction of hydrocarbons. Despite higher water intensity (the amount of water used to produce a unit of energy; for example, liters per gigajoules) of hydraulic fracturing compared to conventional vertical oil and gas wells, it has been shown that the overall water withdrawal for hydraulic fracturing is negligible compared to other industrial water uses on a national level (6, 7, 22, 23). On a local scale, however, water use for hydraulic fracturing can cause conflicts over water availability, especially in arid regions such as western United States, where water supplies are limited (2, 20, 24, 25).
The wastewater generated from hydraulic fracturing is composed of a blend of returned injected hydraulic fracturing water and typically high saline formation water that flows back out of the well after hydraulic fracturing to generate flowback and produced (FP) waters. Over time, the contribution of the saline formation water increases, which results in an increase in the salinity of the FP water. The salts, toxic elements, organic matter, and naturally occurring radioactive material in the FP water pose contamination risks to local ecosystems from spills (14, 26) and mismanagement (6, 27–29). In addition to these risks, treatment of the FP water to safely return and release to the environment is energy-intensive and expensive; thus, many operators are forced to either recycle the FP water onsite for future hydraulic fracturing operations or reinject it into deep-injection wells.
Current technological limitations to the efficiency of hydraulic fracturing include a rapid decrease (20 to 50% of total production after the first year) in unconventional gas and oil production through time after initial production, and the fact that a significant portion of the gas in the shale formations is left unproduced (22, 30). Despite these limitations, advancements in hydraulic fracturing and horizontal drilling technology have increased production of gas and oil from shale regions; between 2007 and 2016, the shale gas production has increased by eightfold in the United States (1). Two recent studies have suggested that intensification of the hydraulic fracturing process through drilling longer horizontal laterals has resulted in increased water use and hydrocarbon production (20, 25). Given the relatively long history of hydraulic fracturing in United States, understanding how the water footprint of hydraulic fracturing has evolved through time with technological advancements and shifting economic conditions is critical (22, 23). Lessons learned from U.S. production development can directly influence planning and implementation of hydraulic fracturing practices, as other countries such as China bring their natural gas reserves online.
For the first time, this study presents systematic temporal data on water use, unconventional shale gas and tight oil production rates, and volume of FP water from major shale-producing regions in the United States. In addition, we combine several databases to estimate the efficiency of production from both hydrocarbons and water perspective on a year-by-year basis, showing that the water footprint of hydraulic fracturing has been steadily increasing through time.
RESULTS
In each of the six regions studied in this report, water use per well is increasing (Fig. 2 and tables S1 and S2). The Marcellus region (Pennsylvania and West Virginia) had the lowest increase in water use (20%), from a median value of 23,400 m3 per well in 2011 to 27,950 m3 per well in 2016, while the Permian Basin (Texas and New Mexico) had the largest increase in water use (770%), from 4900 m3 per well in 2011 up to 42,500 m3 per well in 2016. Median water-use volumes varied largely among regions, with the Bakken region using the least water (21,100 m3 per well in 2016) and the Permian basin using the most water (42,500 m3 per well in 2016). One exception is the Haynesville region, where water use per well decreased with time (Fig. 2). Horizontal drilling requires producers to drill vertically to a target depth and then curve the well horizontally through shale formations, maximizing the surface area producing oil and gas. The length of the portion of the well that was drilled horizontally is referred to as the lateral length, with hydraulic fracturing events occurring in stages as a well is drilled further horizontally. Over the period of 2011–2016, the median length of lateral section of horizontal wells also increased (tables S1 and S2), most likely due to technological development and economic considerations to increase the extraction yields from individual wells. We show below that the hydrocarbon extraction intensity has similarly increased during this period. Parallel to the increase in lateral lengths of the horizontal wells and hydrocarbon extraction yields through time, the water use has also increased. The relative increase in lateral length (0 to 80%) was, however, significantly lower than the increase in water use (14 to 770%). There are two exceptions to this observation: (i) in the Marcellus region, the increase in lateral length (20%) was equal to the increasing water use (25%); (ii) oil-producing wells in the Permian basin, where lateral length increase (79%) and water-use increase (770%) were both higher than those in the other studied regions. When water use per well is normalized to the length of lateral section of the horizontal well, in almost every case, we observed an increase in water use per length of the horizontal well. This pattern is most evident in the Permian region, where water use increased from 4.3 m3per meter in 2011 to 29.0 m3 per meter in 2016 for gas-producing wells, and from 3.9 m3 per meter in 2011 to 19.0 m3 per meter in oil-producing wells (tables S1 and S2). The smallest observed changes were in the Marcellus and Haynesville regions, where water use per horizontal length has been relatively consistent through time.
In all cases, the FP water generation was also increasing through time, with particularly higher rates after 2014 (Fig. 3 and tables S1 and S2). Both the gas- and oil-producing portions of the Eagle Ford region showed large increases through time, with a 610% increase in FP water in the oil-bearing section (from 2400 m3 per well in 2011 to 16,900 m3 per well in 2015) and a 1440% increase in the gas-bearing section (from 1340 m3 per well in 2011 to 20,700 m3 per well in 2015). The smallest increase in FP water occurred in the Niobrara region, where production increased from 1800 m3 per well in 2011 to 2300 m3 per well in 2016.
Fig. 3 - Oil, gas, and FP water variations with time.
Annual shale gas (A), tight oil (C), and FP water (B and D) productions in shale gas–producing regions (A and B) and oil-producing regions (C and D). Whiskers on the bar graphs represent 95% bootstrap confidence intervals (table S1).
Coupled with the increase in water use and FP water production rates, unconventional natural gas production shows an upward trend in production, with volumes increasing through time among the regions. Year 1 shale gas production in the Permian region increased from 10.9 × 106 m3 per well in 2011 to 25.1 × 106 m3 per well in 2016, a 360% increase (Fig. 3). Similarly, year 1 shale gas production in the Marcellus formation increased through time, from 21.8 × 106 m3 per well in 2011 to 29.0 × 106 m3 per well in 2015, before falling to 20.8 × 106 m3 per well in 2016. In contrast, in the Eagle Ford formation, year 1 production remained relatively constant from 2011 to 2014, before increasing from 2014 to 2015.
Unconventional oil production shows a consistent increase in year 1 oil production volume per well through time, with values falling only in the Eagle Ford region from 2014 to 2015. The largest increase was in the Permian region, where oil production increased from 8200 m3 per well in 2011 to 16,700 m3 per well in 2016, a 130% increase (Fig. 3). When comparing our estimate of total year 1 hydrocarbon production (year 1 estimate multiplied by well count estimate) to total hydrocarbon production reported by the Energy Information Administration (EIA) (fig. S1) (31), we see that year 1 production parallels total oil production in most regions in the United States.
We define the water-use intensity for hydraulic fracturing as the amount of water used for hydraulic fracturing to generate a unit of energy from the produced gas and oil (see Materials and Methods) (19, 23, 32). In gas-producing regions, water-use intensity (for the first 12 months of production) ranges from 7 liters/GJ (Haynesville) to 21 liters/GJ (Marcellus) in 2011 and grew to between 13.5 liters/GJ (Haynesville) and 33 liters/GJ (Permian) in 2016 (Fig. 4 and tables S1 and S2). Unconventional oil regions also have increasing water-use intensities, increasing from 11 liters/GJ (Permian) in 2011 to between 28 liters/GJ (Bakken and Permian) in 2016 and 50 liters/GJ (Eagle Ford) in 2015. For comparison, the average water intensity of conventional natural gas is only 4 liters/GJ for drilling and extraction, while coal mining constitutes a mean value of 43 liters/GJ (fig. S4) (33). Water-use intensity is also calculated as the ratio between the volume of water used and the volume of hydrocarbon produced (tables S1 and S2 and fig. S2). The increase of the water use to hydrocarbon production ratios with time indicates that the intensification of hydraulic fracturing process to increase hydrocarbon production rates involves net addition of water, and thus, the water intensity has increased with time.
Fig. 4 - The changes in the water intensity of hydraulic fracturing with time.
Water-use intensity variations with time for hydraulic fracturing of shale gas (A) and tight oil (C) regions and corresponding FP water/water use ratios in shale gas (B) and tight oil (D) regions. Water-use intensity is defined as the amount of water required to generate a unit of energy. (A) and (B) show the water-use intensity for shale gas–producing regions, while (C) and (D) show water-use intensity for unconventional oil-producing regions. Whiskers represent 95% bootstrap confidence intervals.
When comparing the volume of FP water production rates to the water used for hydraulic fracturing, we show that, in many cases, more water is used for hydraulic fracturing than returns as FP water over the first year (Fig. 4; FP water/water use ratio < 1). In shale gas–producing regions, we see an increase in the ratio through time, with the exception of the Permian region, which increases from 2011 to 2014 but then drops in 2015 and 2016. The Permian region is also unique as the FP water/water use ratios for both unconventional oil and gas wells are higher than 1. Unconventional oil-producing regions have varying trends in the FP water/water use ratio. Wells in the Eagle Ford oil region have an increasing ratio through time, while no consistent trend is observed in the Bakken, Permian, and Niobrara regions (Fig. 4 and table S2). Regions where the FP water/water use ratios were increasing through time present a growing water management challenge, as the net increase (14 to 770%) in the water use (Fig. 4 and tables S1 and S2) is coupled with increasing FP water production, growing at even higher rates (60 to 1440%). This trend exacerbates water management issues because producers must now manage increasingly large volumes of water for hydraulic fracturing operations as well as larger oil and gas wastewater volumes that need to be adequately disposed.
DISCUSSION
Much of the controversy surrounding hydraulic fracturing revolves around the use of large volumes of water to hydraulically fracture wells. Concern is especially high in semiarid regions (Fig. 1), where water withdrawals for hydraulic fracturing can account for a significant portion of consumptive water use within a given region, even contributing to groundwater resource depletion (2). Overall, there have been calls to increase the use of alternative water sources such as brackish water or recycling FP water, minimizing the strain on local freshwater resources (2, 25).
Previous studies have suggested that hydraulic fracturing does not use significantly more water for exploration and production than other energy sources (fig. S4) and, at the same time, indicated that water use for hydraulic fracturing makes up only a small fraction of the industrial water utilization in the United States (7, 22, 23, 33). These evaluations were based on aggregated water footprint data during the early years (2011–2014) of hydraulic fracturing in the United States. Here, we show, however, steadily increasing volumes of water use with time in all the major unconventional gas and oil regions (Fig. 2 and tables S1 and S2). Parallel to the increase of shale gas and tight oil production intensity, we also show that the water intensity of hydraulic fracturing is increasing for both unconventional gas and oil regions (Fig. 4 and tables S3 and S4). In addition, the water used for hydraulic fracturing is retained within the shale formation; only a small fraction of the fresh water injected into the ground returns as flowback water, while the greater volume of FP water returning to the surface is highly saline, is difficult to treat, and is often disposed through deep-injection wells. This means that despite lower water intensity compared to other energy resources (fig. S4), the permanent loss of water use for hydraulic fracturing from the hydrosphere could outweigh its relatively lower water intensity.
The period of 2014–2015 marks a turning point, where water use and FP water production began to increase at higher rates. During this period, gas and oil prices dropped significantly, causing producers to scale back the number of new installed wells (Fig. 5 and tables S1 and S2). In each of the oil-producing regions, the water use/oil production ratio increased, suggesting that the increase in water use for hydraulic fracturing outpaces the increasing oil production on a per-well basis (Fig. 4, fig. S2, and table S2). In the shale gas–producing regions, this trend is also present, but not as strongly apparent as with the unconventional oil-producing regions (Fig. 4, fig. S2, and table S1). Consequently, while increasing lateral length of horizontal drilling and water use for hydraulic fracturing (Fig. 2) have resulted in increasing oil production (per well), the net water-use efficiency, particularly for unconventional oil production, has decreased (that is, higher water intensity).
Fig. 5 - New shale gas and tight oil well installations compared to oil and gas prices.
Variations of installed well counts (left y axis) and gas and oil prices (right y axis) with time for shale gas–producing regions plotted with corresponding natural gas citygate price (top) and for oil-producing regions with corresponding crude oil price (bottom). The data show the number of new well installations corresponding closely with the contemporary gas and oil prices. MCF, thousand cubic feet; BBL, barrel.
By combining the increasing trends in both water use and FP water production with the increasing FP water/water use ratios in some regions, we can see that the overall water footprint of hydraulic fracturing is increasing through time; more water is being used for hydraulic fracturing operations, while, at the same time, comparatively more FP water is being generated. We observed increasing total water use (water use per well multiplied by well count; fig. S3) in oil-producing regions despite the recent slowdown in oil production rates (Fig. 5). Assuming that the recent economic downturn eventually subsides and the drilling of new wells again reaches levels seen during the heyday of hydraulic fracturing in the early 2010s, the total water impact of hydraulic fracturing is poised to increase markedly in both shale gas– and oil-producing regions. On the basis of modeling future hydraulic fracturing operations in the United States in two scenarios of drilling rates, we project cumulative water use and FP water volumes to increase by up to 50-fold in unconventional gas-producing regions and up to 20-fold in unconventional oil-producing regions from 2018 to 2030, assuming that the growth of water use matches current growth rates and the drilling of new wells again matches peak production (fig. S5 and tables S3 and S4). Even if future drilling rates will stay at 2016 levels (that is, low oil gas prices), we predict a large increase of the total water use for both unconventional oil and shale gas basins (fig. S5). Likewise, we predict a large increase in the FP water volume for the two scenarios, with particularly high total FP water production in the Permian and Eagle Ford basins (fig. S5).
The increase in the water footprint of hydraulic fracturing shown in this study has serious implication for local communities, where increased drilling volume will lead to large instantaneous water demands, and resulting in increasing FP water burdens that will have to be managed into the future. The predicted increasing water use and FP water production in the Permian and Eagle Ford basins are alarming given the extreme water scarcity in these regions (Fig. 1). The results presented in this study are consistent with previous studies in the Permian (19) and Eagle Ford (25) basins that have shown that local water resources could be affected by increasing water demands for hydraulic fracturing. At the same time, other studies have shown that water use for hydraulic fracturing in water-rich areas such as the Sichuan basin in China (8, 34) will constitute only a small fraction of the available local water resources. While the water intensity during early stages of hydraulic fracturing (<30 liters/GJ in most cases) was comparable and even lower than that of coal mining (43 liters/GJ; fig. S4), the recent (2014–2017) intensification of hydraulic fracturing has increased the water intensity, particularly for unconventional oil (up to 93 liters/GJ; fig. S4). Additional studies are needed to analyze the local impacts of hydraulic fracturing on water resource depletion in light of increasing water demand for hydraulic fracturing and the increasing volumes of FP water that need to be managed, particularly in areas vulnerable to induced seismicity from injection of large volumes of oil and gas wastewater. As unconventional gas and oil exploration is expanding globally and other countries begin to follow the U.S. shale revolution (for example, China) (34), the results of this study should be used as a guidance for the expected water footprint of hydraulic fracturing at different stages of energy development.
MATERIALS AND METHODS
Experimental design
The goal of this study was to synthesize and collate production volumes for unconventional gas and oil production, associated FP water, and water use for hydraulic fracturing per shale gas/oil well. We downloaded production data for each of these components from the major shale gas and tight oil regions identified in this study using multiple sources and separated by year of initial production. Once organized, data were reported based on the first 12 months of the well lifetime to see how production and water-use values compared on a year-by-year basis.
Data acquisition
Production volumes for gas, oil, and FP water for each reporting well in our target regions were downloaded using the DrillingInfo Desktop application (35). Data were downloaded by primary well production type (gas or oil). FP water data (and natural gas production for 2015–2016) were not available from the Marcellus region, and thus, data from the Marcellus Formation were downloaded from the gas and oil reporting website of the Pennsylvania Department of Environmental Protection (PA DEP) (36). Water-use data were downloaded from the FracFocus Chemical Disclosure Registry (37). Well counts from 2014 to 2016 were taken from the EIA Drilling Productivity Report (DPR) (31), while well counts from 2012 to 2014 were taken from Baker Hughes’ onshore well counts (38). The well counts provided did not indicate if they were taken from conventional or unconventional oil-producing wells or in regions producing both gas and oil, and whether the well was classified as an oil well or gas well. Consequently, we used DrillingInfo to find the ratio of conventional to unconventional wells and the ratio of oil to gas wells for each region for each year and multiplied that by the number of wells reported by Baker Hughes and in the DPR. In addition, wells drilled in the Bakken formation were not reported by the Baker Hughes report. As a result, Bakken Formation well counts from 2011 to 2013 were taken from the North Dakota Oil and Gas Division (39). Combining data from several resources can introduce bias and error into results using these values. Values reported in this study were found to be comparable to those reported in previous studies. Natural gas and oil historical prices were taken from the EIA (1).
Statistical methods
Data from over 12,000 individual wells were organized by producing region, American Petroleum Institute (API) number, first production year, and month since first production. Data were sorted by first production year and then aligned within each year by month since first production. The median values among all API numbers reporting data within a given year were calculated for each month since production began (22, 23). A similar analysis was done with the PA DEP Marcellus data. Finally, areas where less than 0.5% of data are reported (number of DrillingInfo reports/well count < 0.5%) were removed from the data set. This removed DrillingInfo data for the Haynesville formation from 2015 and 2016, the Eagle Ford gas- and oil-producing regions from 2016, and the Marcellus formation from 2015 to 2016. Marcellus data from 2015 to 2016 were replaced with data from PA DEP. The region for each water-use data point was determined spatially using the latitude and longitude provided by FracFocus to locate points within unconventional gas and oil plays (shapefile provided by the U.S. EIA) (31). Water-use data were then sorted by region, and the median water-use value was calculated for each spud year.
Energy intensities were calculated by converting production volumes of gas and oil to energy production using their energy content. Energy content for natural gas was assumed to be 0.038 and 38.18 GJ/m3 for oil (2). Calculations for gas-producing wells also included associated oil production with an energy content of 85% that of crude oil, or 32.45 GJ/m3, as well as the shrinkage of 25% of the wet gas stream to natural gas liquids with an energy content of 25.54 GJ/m3 (2, 23, 32). For oil wells, associated gas production with an energy content of 0.038 GJ/m3 was added.
Bootstrap confidence intervals were used to estimate variation in water use, gas production, oil production, and FP water production (22, 40). For each data set, 95% bootstrap confidence intervals of the median for each month of production were calculated. The first 12 months for each data set were added together to form year 1 estimates. We drew bootstrap resamples with replacement from each month’s empirical distribution. This was repeated 1000 times, and distributions were drawn from the 0.025 and 0.975 percentile values (22). In cases where data with errors were used to calculate new terms, error was propagated through the calculation.
Production predictions
Cumulative future water use (and year 1 production) was defined as the number of wells drilled in a given year and region, multiplied by the water use per well (plus year 1 FP water production). We outlined two scenarios to predict cumulative future water use and year 1 production. The first was a business-as-usual scenario, where we assumed that the number of new wells drilled each year remained constant from 2018 to 2030 and was equal to the number of new wells drilled in 2016 in each formation. We then multiplied this well count by an estimate of the future water use per well (or year 1 FP water per well). Future water use per well and future year 1 FP water predictions were made using the maximum respective increases from 1 year to another for each formation (Figs. 2 and 3 and tables S1 to S4). This calculation yielded a linear increase in cumulative water use and year 1 FP water through time (figs. S5 and S6). A second scenario was created to predict the results of a return to previously high drilling rates (that is, high oil prices). Our calculation for future water use per well and future year 1 FP water remained the same. To estimate the number of new wells drilled each year, we calculated the slope of the largest increase in well count from 1 year to another for each region and added that slope to 2016 well counts, resulting in a linear increase in the number of new wells drilled each year. This resulted in a parabolic increase in cumulative future water use through time (figs. S5 and S6).ΩThis is an open-access article distributed under the terms of the Creative Commons Attribution-NonCommercial license, which permits use, distribution, and reproduction in any medium, so long as the resultant use is not for commercial advantage and provided the original work is properly cited.
____________________________
- Copyright © 2018 The Authors, some rights reserved; exclusive licensee American Association for the Advancement of Science. No claim to original U.S. Government Works. Distributed under a Creative Commons Attribution NonCommercial License 4.0 (CC BY-NC)
Comments
Be the first to comment